Source: http://www.humanneurophysiology.com/autonomicns.htm
Fortunately the body's vital functions are regulated automatically and require no conscious effort on our part. The autonomic nervous system (ANS) is to a large extent responsible for automatically and subconsciously regulating the cardiovascular, renal, gastrointestinal, thermoregulatory, and other systems, in order to enable the body to meet the continual and ever-changing stresses to which it is exposed.
Autonomic nerve fibers innervate cardiac muscle, smooth muscle, and glands. Through these fibers the ANS plays a role in regulating (1) blood pressure and flow, (2) gastrointestinal movements and secretions, (3) body temperature, (4) bronchial dilation, (5) blood glucose levels, (6) metabolism, (7) micturition and defecation, (8) pupillary light and accommodation reflexes, and (9) glandular secretions, just to name a few.
A muscle or gland innervated by autonomic fibers is called an effector organ. If the autonomic nerve fibers to an effector organ are cut, the organ may continue to function, but will lack the capability of adjusting to changing conditions. If the autonomic nerve fibers to the heart are cut, the heart will continue to beat and pump blood normally, but its ability to increase cardiac output under stress will be seriously limited. In a very real sense, the ANS bestows on the vital functions of the body the capability of adjusting activity levels to meet ever-changing needs.
Anatomically and functionally, the autonomic nervous system is made up of two subdivisions: the sympathetic system with long-lasting and diffuse effects, and the parasympathetic system with more transient and specific effects. In either case the nerve fibers of the ANS are motor only, and represent the general visceral efferent (GVE) fibers of the cranial and spinal nerves.
THE SYMPATHETIC OUTFLOW
The nerve fibers which comprise the sympathetic system originate in the intermediolateral horn (lamina VII) of the gray matter in all twelve thoracic and the first two lumbar segments of the spinal cord. The axons of these GVE fibers travel through the anterior horn and exit the cord in the anterior root before entering the spinal nerve. While the general somatic efferent (GSE) fibers (alpha and gamma motor neurons of the anterior horn) continue in the spinal nerve trunks to innervate skeletal muscle fibers and muscle spindles, almost all of the GVE fibers leave the spinal nerve trunks to enter sympathetic ganglia via a thin arm, the white ramus (Figs-1, 2, and 3).
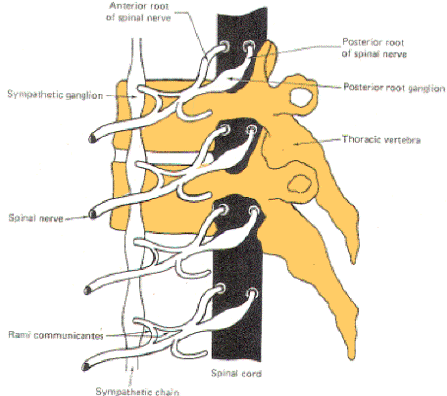 | 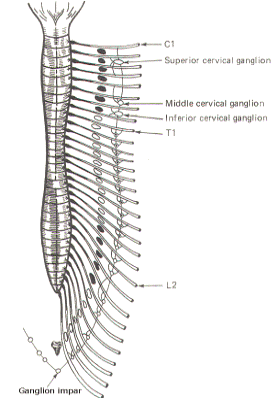 | 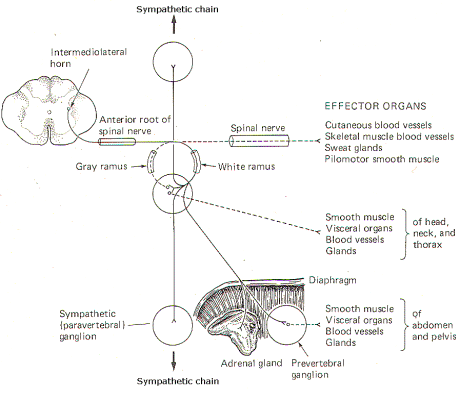 |
Fig-1: The sympathetic ganglia associated with spinal nerves T1 through L2 are connected to the nerve by two arms, the rami communicantes albicans and gresium | Fig-2: There are no rami albicans above T1 and below L2. The gray rami send connections to all 31 nerves. | Fig-3: The sympathetic outflow. The preganglionic neurons originate in the intermediolateral horn of the spinal cord, between T1 and L2 |
The sympathetic ganglia lie close to the vertebral bodies and are also known as paravertebral ganglia. They are strung together to form a sympathetic or paravertebral chain. There are two of these chains, one on either side of the vertebral column connected in front of the coccyx by the single ganglion impar (Fig-2).
Some of the fibers from nerve cells within the ganglia return to the spinal nerve trunk via a gray ramus. The fibers traveling through the white rami are myelinated while those in the gray rami are not, and this fact is responsible for their respective names. Each of the twelve thoracic and first two lumbar nerves is in contact with a paravertebral ganglion via a white and gray ramus. However, there are three ganglia in the chain above the thoracic region as well as several below L2 (Fig. 14-2). Each of these additional ganglia is connected to a spinal nerve by a single gray ramus.
The superior, middle, and inferior cervical ganglia probably represent the fusion of smaller individual cervical ganglia. These three send gray rami to all eight cervical spinal nerves. The superior cervical ganglion sends to the first four cervical nerves, the smaller middle cervical ganglion supplies the next two, and the large inferior cervical ganglion projects a gray ramus to the seventh and eighth cervical nerves. Similarly, a variable number of ganglia (four to eight) below L2 send gray rami to all of the spinal nerves below this level. Consequently, all 31 pairs of spinal nerves are in contact with the sympathetic chain and carry fibers of the sympathetic system. This is an important feature, enabling those effector organs which are innervated only by spinal nerves (cutaneous and skeletal muscle blood vessels, sweat glands, and pilomotor smooth muscle) to receive sympathetic input.
In addition to the paired paravertebral ganglia, there are several unpaired prevertebral ganglia in the abdomen and pelvis. They also playa role in the sympathetic outflow. Figure 14-3 illustrates the many possible ways by which the sympathetic system innervates its effector organs.
There is always a two-neuron link to each effector organ innervated. with the single exception of the adrenal medulla. The first is the preganglionic neuron and the second is the postganglionic neuron. The four possible routes of the preganglionic and postganglionic fibers, as illustrated in Fig. 14-3, are summarized below. After entering the sympathetic ganglia via the white rami, the preganglionic fibers may:
1 Pass without synapsing up or down the sympathetic chain to ultimately synapse in a higher or lower ganglion. By passing up the chain, the first four or five thoracic cord levels contribute all of the preganglionic fibers to the superior, middle, and inferior cervical ganglia. Similarly by passing down the chain, the lower thoracic and upper lumbar cord levels contribute all of the preganglionic fibers to the ganglia in the chain below L2. Postganglionic fibers then leave the ganglia via their gray rami to enter their respective spinal nerves for distribution to their effector organs (cutaneous and skeletal muscle blood vessels. sweat glands, and pilomotor smooth muscle).
2 Synapse in the ganglia and subsequently stimulate postganglionic fibers which leave the ganglia to reenter the spinal nerves via the gray rami. The postganglionic fibers are then distributed with the spinal nerves to their effector organs (cutaneous and skeletal muscle blood vessels. sweat glands, and pilomotor smooth muscle).
3 Synapse in the ganglia and subsequently stimulate postganglionic fibers which leave the ganglia and are directly distributed to their effector organs (smooth muscle, visceral organs. blood vessels, and glands of the head, neck, and thorax).
4 Pass without synapsing into the abdomen to synapse in one of the prevertebral ganglia or the adrenal medulla. Postganglionic fibers leave the prevertebral ganglia to innervate their effector organs (smooth muscle. visceral organs. blood vessels, and glands of the abdomen and pelvis).
 |
THE PARASYMPATHETIC OUTFLOW
The nerve fibers which comprise the parasympathetic system originate in two quite distant regions, the brain stem and the sacral portion of the spinal cord. For this reason it is often called the craniosacral outflow to distinguish it from the thoracolumbar outflow of the sympathetic system. Those GVE fibers which make up the cranial portion of the system originate in specific brain stem nuclei and are distributed with cranial nerves III, VII, IX, and X. Those which comprise the sacral portion originate in lamina VII of sacral cord segments 2 to 4 and are distributed as the GVE fibers of the pelvic nerves (nervi erigentes). As with the sympathetic system, there are always two neurons in the pathway to the effector organ supplied. Thus, there are pre- and postganglionic fibers in the parasympathetic system also. However, unlike those in the sympathetic system, parasympathetic ganglia are quite distant from the brain stem and cord, often located directly on the effector organ itself. Thus the postganglionic fibers are much shorter in the parasympathetic system than they are in the sympathetic system. It should be noted here that autonomic effector organs typically receive both sympathetic and parasympathetic innervation, though some receive input from one system only. The effects of sympathetic and parasympathetic stimulation of the autonomic effector organs are summarized in Table-1. The effects are often but not always opposite, as will be described later.
Figure-4 illustrates the parasympathetic outflow. The EdingerWestphal nucleus (an accessory nucleus of III) in the tegmentum of the midbrain gives rise to the preganglionic parasympathetic fibers of the oculomotor (III) nerve. Some of these fibers terminate in the ciliary ganglion and others in the episcleral ganglion. The former stimulate postganglionic fibers innervating the sphincter muscles of the iris, which control pupillary diameter, while the latter stimulate postganglionic fibers innervating the ciliary muscle controlling the curvature of the lens (Fig-5).
The superior salivatory nucleus in the pons gives rise to the preganglionic fibers of the facial (VII) nerve. Some of these fibers terminate in the sphenopalatine ganglion and others in the submandibular (submaxillary) ganglion. Postganglionic fibers from the former innervate the lacrimal gland and mucous membranes in the head and neck region while postganglionic fibers from the latter innervate the submaxillary and sublingual salivary glands. The inferior salivatory nucleus at the pontomedullary border gives rise to the preganglionic fibers of the glossopharyngeal nerve (IX). These fibers terminate in the otic ganglion, from which postganglionic fibers innervate the parotid gland.
The overwhelming majority of cranial preganglionic fibers are distributed within the vagus (X) nerve. They originate in the dorsal motor nucleus of X in the medulla and terminate in unnamed peripheral ganglia on thoracic and abdominal organs, glands, and some blood vessels. Short postganglionic fibers run from these ganglia to receptor sites on the effector organ cells.
The sacral parasympathetic outflow supplies the organs and glands in part of the lower abdomen and all of the pelvis. Included are the descending colon, sigmoid, rectum, bladder, and external genitalia. As noted earlier, the preganglionic fibers originate in lamina VII of the sacral cord between S2 and S4, These fibers travel with the pelvic nerve and terminate in peripheral ganglia on the effector organs themselves.
|
Fig-4: The parasympathetic outflow
| |
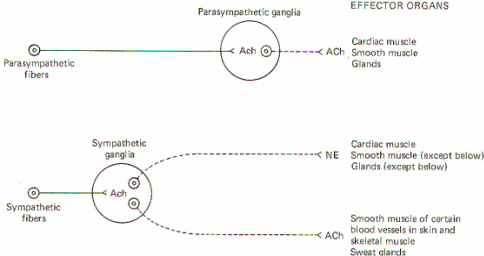 |
AUTONOMIC NEUROTRANSMITTERS
Both sympathetic and parasympathetic preganglionic neurons are cholinergic; that is, the preganglionic fibers of both systems release acetylcholine (ACh) at the synapse in the ganglion. Thus ACh is the principal transmitter in the autonomic ganglia. There are also some dopaminergic (dopamine releasing) interneurons present, but their function is still unknown. Nevertheless, the preganglionic fibers themselves are all cholinergic.
All postganglionic fibers of the parasympathetic system are cholinergic, but postganglionic sympathetic fibers are more diverse. The overwhelming majority areadrenergic [release norepinephrine (NE)], but a few are cholinergic. The few which are known to be cholinergic are those which innervate the sweat glands and some cutaneous and skeletal muscle blood vessels. (Fig-5)
|
Fig-5: General scheme of autonomic neurotransmitters.
| |
Acetylcholine Synthesis, Release, and Inactivation
Figure-6 illustrates the general scheme of activity at the cholinergic synapse. Synthesis of ACh occurs in the cytoplasm of cholinergic presynaptic terminals. Coenzyme A (CoA) combines with acetate to form acetyl coenzyme A (acetyl CoA). Energy for this reaction is supplied by ATP. Once formed, the acetyl CoA combines with choline in the presence of the enzyme choline acetyltransferase to form acetylcholine (ACh). Once synthesized, ACh is taken up by the synaptic vesicles and held there in a bound form until its released.
When an impulse reaches the presynaptic terminal, several synaptic vesicles release ACh into the synaptic cleft. ACh then diffuses across the cleft to activate cholinergic receptor sites on the postsynaptic membrane. In order to allow the presynaptic terminal to effectively control the postsynaptic membrane, the released ACh must be quickly degraded (within microseconds) by the enzyme acetylcholinesterase (AChE) to acetate and choline, which are then reabsorbed into the presynaptic terminal for resynthesis to ACh. A small fraction is reabsorbed intact into the presynaptic terminal while an even smaller fraction diffuses out of the synaptic cleft before it can be degraded or reabsorbed. AChE is abundantly available in the cholinergic synaptic cleft. And even though the enzyme can degrade ACh within microseconds, there is adequate time for the ACh to activate receptor sites.Norepinephrine Synthesis, Release, and Inactivation.
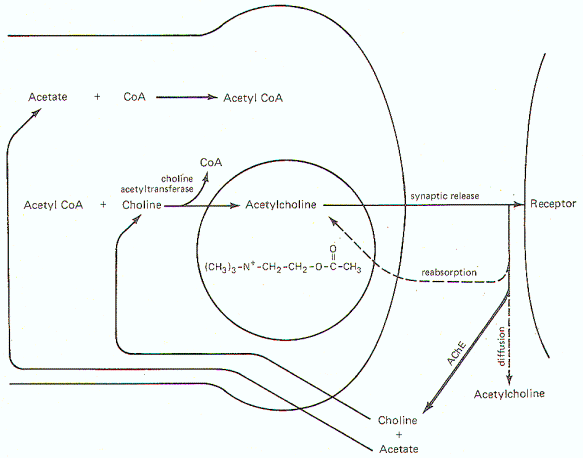 | 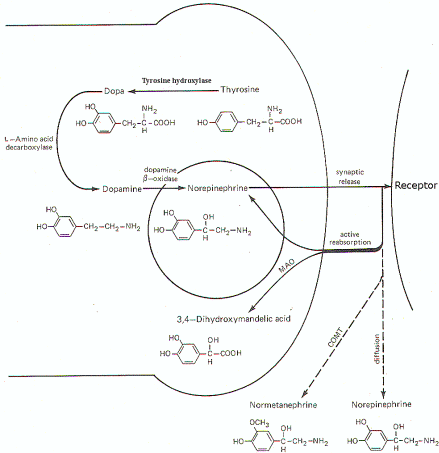 |
Fig-6: Synthesis and fate of synaptically released acetylcholine at cholinergic synapse. | Fig-7: Synthesis and fate of synaptically released norepinephrine at adrenergic synapse. |
Figure-7 illustrates the synthesis and fate of synaptically released norepinephrine at adrenergic synapses. Norepinephrine is synthesized in the presynaptic terminal by a series of enzymatically catalyzed reactions typically starting with the amino acid tyrosine. The sequence can also start with phenylalanine, which can be enzymatically converted to tyrosine. In either case tyrosine is converted to dihydroxyphenylalanine (dopa), dopamine, and finally to norepinephrine. The final synthetic step from dopamine to norepinephrine occurs in the synaptic vesicle where the norepinephrine is held in a bound form. The formation of dopa is apparently the rate-limiting step in the synthesis of norepinephrine. When an impulse reaches the presynaptic terminal, several vesicles release norepinephrine into the synaptic cleft, where it diffuses to activate receptor sites on the postsynaptic membrane. Within a few milliseconds, the norepinephrine is subject to one of three fates. A small amount is methylated by the enzyme catechol-o-methyl transferase (COMT), which is present in the cleft, and thereby rendered inactive. An even smaller fraction diffuses out of the cleft and away from receptor sites. But certainly the greatest amount of norepinephrine is reabsorbed by active transport into the presynaptic terminal. If norepinephrine stores in the synaptic vesicles are low, as might be the case in a rapidly firing fiber, the reabsorbed norepinephrine may be taken up by the vesicles for subsequent rerelease. If adequate stores of the transmitter are available, the reabsorbed norepinephrine is subjected to oxidative deamination by mitochondrial monoamine oxidase (MAO).
AUTONOMIC TONE
Table1 shows the effects of sympathetic and parasympathetic stimulation on autonomic effector organs. The sympathetic and parasympathetic systems are continually active and the level of activity at a given rate of firing is known as autonomic tone.
Table-1 Autonomic Effects on Various Organs of the Body
|
Effector organs | Effects of sympathetic stimulation | Effects of parasympathetic stimulation |
Eye | Radial muscle of the iris | (α) Contraction (mydriasis) | |
| Sphincter muscle of the iris | | Contraction (myosis) |
| Ciliary muscle of the lens | (β) Relaxation Lens flattens | Contraction ( Lens curves) |
Heart | SA node | (β) ↑ heart rate | ↓ heart rate |
| Atria | (β) ↑ heart rate and force | ↓ heart force |
| AV node | (β) ↑ conduction velocity | ↓ conduction velocity |
| Purkinje system | (β) ↑ conduction velocity | |
| Ventricles | (β) ↑ heart rate and force | |
Blood vessels | Coronary | (α) Constriction | Dilatation |
| | (β) Dilatation | |
| Cutaneous | (α) Constriction | |
| | (ACh) Dilatation | |
| Skeletal muscle | (α) Constriction | |
| | (β) Dilatation | |
| | (ACh) Dilatation | |
| Abdominal visceral | (α) Constriction | |
| | (β) Dilatation | |
| Renal | (α) Constriction | |
| Salivary glands | (α) Constriction | Dilatation |
Stomach | Motility and tone | (β) Decrease (usually) | Increase |
| Sphincters | (α) Contraction (usually) | Relaxation (usually) |
| Secretion | Inhibition (?) | Stimulation |
Intestine | Motility and tone | (α, β) Decrease | Increase |
| Sphincters | (α) Contraction (usually) | Relaxation (usually) |
| Secretion | Inhibition (?) | Stimulation |
Gallbladder and ducts | | Relaxation | Contraction |
Urinary bladder | Detrusor | (β) Relaxation (usually) | Contraction |
| Trigone and sphincter | (α) Contraction | Relaxation |
Ureter | Motility and tone | Increase (usually) | Increase (?) |
Male sex organs | | Ejaculation | Erection |
Skin | Pilomotor muscles | (α) Contraction | |
| Sweat glands | (α) Slight, localized secretions | |
| | (ACh) Generalized secretions | |
Spleen capsule | | (α) Contraction | |
Lung (bronchial muscles) | | (β) Relaxation | Contraction |
Adrenal medulla | | | Secretion of epinephrine and norepinephrine |
Liver | | (β) Glycogenolysis | |
Pancreas | Acinar cells | ↓ secretion | Secretion |
| Islet cells | (α) Inhibition of insulin and glucagon secretion | Insulin and glucagon secretion |
| | (β) Insulin and glucagon secretion | |
Salivary glands | | (α) Thick, sparse secretion | Profuse, watery secretion |
Lacrimal glands | | | Secretion |
Nasopharyngeal glands | | | Secretion |
Adipose tissue | | (β) Lipolysis | |
Juxtaglomerular cells | | (β) Renin secretion | |
Pineal gland | | (β) Melatonin synthesis and secretion | |
Sympathetic Tone
To illustrate sympathetic tone, consider this example. Most arteries are normally in a state of partial constriction. That is, they are neither fully constricted nor fully dilated. Since most blood vessels receive only sympathetic innervation, it is the only system that need be considered. If the normal partially constricted state of an artery is maintained by a basal firing rate of 1 impulse per second, we can describe the artery as displaying a basal sympathetic tone. Now if the firing rate should increase to say 50 impulses per second, the artery would constrict further, showing an increase in sympathetic tone. Conversely, if the firing rate were to decrease, the smooth muscle of the blood vessel would relax, causing the artery to vasodilate with a decrease in sympathetic tone.
The adrenal medulla is also an important contributor to sympathetic tone throughout the body. Each time the sympathetic system is activated, the adrenal medullae are also sufficiently stimulated via the splanchnic nerves, to increase their output of epinephrine and norepinephrine to the general circulation. These two catecholamines then travel to all parts of the body stimulating sympathetic effector organs. It is easy to see how the increased release of these two chemicals by the adrenal medulla can cause a general increase in sympathetic tone throughout the body. In fact. this increased output by the adrenal gland with sympathetic stimulation is the principal reason why the effects of sympathetic stimulation are longer lasting and more diffuse than those associated with the parasympathetic system.
Parasympathetic Tone
An example of parasympathetic tone is the control of peristalsis in the GI tract. Gastrointestinal smooth muscle receives both sympathetic and parasympathetic innervation. Increasing the firing rate of parasympathetic fibers to the gut causes an increase in intestinal motility and peristalsis, and hence, an increase in parasympathetic tone. Decreasing the firing rate produces a decrease in peristaltic activity, and hence, parasympathetic tone. Table-1 shows parasympathetic stimulation increases peristalsis while sympathetic stimulation decreases it. Thus, the GI musculature is an example of the often true observation that the effects of sympathetic and parasympathetic stimulation are opposite and tend to balance each other. Further examination of Table-1 , however, will show that this is not always true.
Alpha and Beta Receptors
The action of catecholamines on adrenergic effector organs varies with the organs. Catecholamines excite some effectors and inhibit others. Experiments with a series of sympathetic drugs have shown there are at least two types of adrenergic receptors. They are called alpha and beta. Blocking agents were later developed for each receptor which further confirmed their existence. The response of an effector to a catecholamine is then partly a function of the type of receptor it has. Epinephrine excites both alpha and beta receptors quite equally, while norepinephrine excites mainly alpha receptors. Nevertheless, norepinephrine will also excite beta receptors, but only to a slight extent. This explains why epinephrine has a much stronger effect on the heart (which has only beta receptors) than norepinephrine does. To further confuse the picture, some effectors have only alpha receptors, others have only beta receptors, and still others have both. Thus the specific response of an effector is both a function of the relative ratio of receptor types and the kind of transmitter involved. A partial list of the effects of alpha and beta stimulation is given in Table-2.
Table-2 Effects of Alpha and Beta Stimulation |
Alpha receptor | Beta receptor |
Vasoconstriction | Vasodilation |
Mydriasis (pupil dilation) | Cardioacceleration |
Intestinal relaxation | Bronchial relaxation |
| Increased cardiac strength |
|
Glycogenolysis
|
| Lipolysis |
Notice that some alpha functions are inhibitory while others are excitatory. The same is true for certain beta effects. Therefore it is not possible to refer to one receptor as excitatory and the other as inhibitory, as is sometimes true. Beta receptors have also been divided into two types: beta1 and beta2, according to their responses to various drugs. Beta1 receptors are those responsible for the inotropic (strength) and chronotopic (rate) responses of the heart. as well as lipolysis. Beta2 receptors bring about vasodilation and bronchial relaxation. This is a distinction useful to the pharmacologist, who can then use a beta2 agonist to treat asthma and produce bronchial relaxation with very little cardiac stimulation.
AUTONOMIC AND RELATED DRUGS
A large number of drugs have been developed which are active at various sites in the autonomic nervous system. Figure 10 schematically illustrates the action and site of action of several of these.
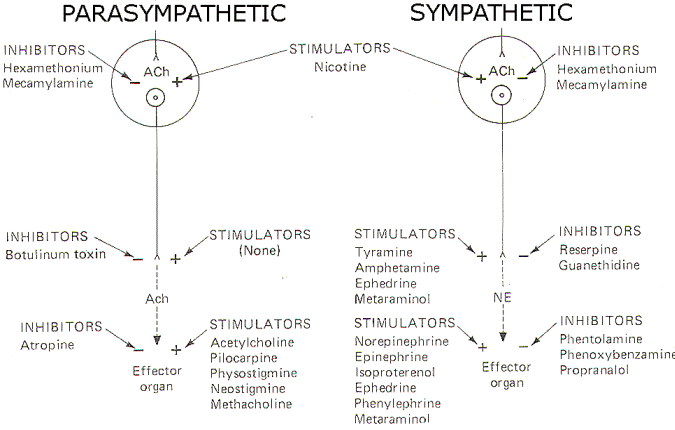
Fig-8: Action of various drugs on the autonomic nervous system.
Drugs Acting on Autonomic Effector Organs
Acetylcholine, pilocarpine, and methacholine all directly stimulate cholinergic receptors on autonomic effector organs. Physostigmine and neostigmine also potentiate activity at these receptors, but do it by the indirect action of inhibiting cholinesterase (AChE). Conversely, atropine is a potent antagonist at these receptors, inhibiting the action of endogenously released ACh as well as administered cholinomimetic drugs.
A variety of drugs are also active at adrenergic receptors on autonomic effector organs. Norepinephrine, epinephrine, isoproterenol (a beta agonist) and phenylephrine (an alpha agonist) are all capable of directly stimulating these receptors. In addition, ephedrine and metaraminol can act directly on these receptors but typically are first absorbed by the adrenergic nerve endings and subsequently released upon the arrival of impulses at the presynaptic terminal. Metaraminol is an alpha agonist both directly and indirectly, while ephedrine is a beta agonist directly but stimulates alpha receptors when released by adrenergic nerve endings. On the other hand, phentolamine and phenoxybenzamine are effective alpha antagonists and thus effectively block alpha receptors. Propranolol is a beta blocker.
Drugs Acting on Autonomic Nerve Endings
There are no known drugs to stimulate the release of ACh from the presynaptic terminals of cholinergic nerve endings. However, botulinum toxin is a potent inhibitor of ACh release. Adrenergic nerve endings are more commonly manipulated by drug action. Both tyramine and amphetamine promote the release of endogenous norepinephrine from these nerve endings. Ephedrine and metaraminol are also potentiators at these sites by the indirect action of being absorbed into the terminals and subsequently being released as false transmitters. Reserpine and guanethidine are effective inhibitors here by the action of depleting stores of norepinephrine in synaptic vesicles and preventing their further uptake and storage.
Drugs Acting on Autonomic Ganglia
Drugs active at parasympathetic ganglia are equally effective at sympathetic ganglia, and vice versa. Nicotine stimulates postganglionic neuron receptors in the autonomic ganglia. Hexamethonium and mecamylamine effectively block these "nicotinic" receptor sites.
Nonautonomic Drugs
It is worth pointing out that there are several drugs which are active at the skeletal neuromuscular junction which are not active in the autonomic nervous system. For example, curare and succinylcholine effectively block the action of ACh on skeletal muscle receptors but have no similar ACh blocking action on cardiac, and smooth muscle receptors.