Alexander C. MettingVanRijn, Antony P. Kuiper, Andre‚ C. Linnenbank and Cornelis A. Grimbergen
Academic Medical Center, Medical Physics Department, Meibergdreef 15 1105 AZ Amsterdam, The Netherlands, e-mail : metting@biosemi.com
- Abstract - A design for patient isolation in 64-channel ECG recordings is presented. Small dimensions of the isolated section and the use of an optical fiber as the only connection between the isolated section and the grounded section of the measurement system, ensured a minimal capacitance between the patient and the environment. The consistent low-power design of the isolated section resulted in a power consumption of 210 mW, which enabled a 10 hour continuous operating time of the battery powered isolated section. The system handles 64 signals with a dynamic range of 75 dB. Analog to digital conversion is performed in the isolated section with a sample rate of 1 kHz per channel. The receiver interfaces to a commercially available DMA board for a standard personal computer.
I. INTRODUCTION
It was argued in a previous paper [4] that the application of isolation amplifiers to transmit the bioelectric signals across the isolation barrier can only form a proper solution in systems with a relatively low number of channels. Given that isolation amplifiers do not offer an optimal solution for multi-channel measurements, we decided to use an optical fiber as the only link between the isolated section and the grounded signal processing section. The power supply of the isolated section remains a problem with the currently available technology. The usual DC-DC converters jeopardize the low isolation capacitance offered by a fiber connection. Optical supply of the needed power by fiber has been reported by some researchers , but the technology is not yet commercially available. We concluded that power supply of the isolated section by batteries is still the best solution. However, in order to make this solution acceptable for daily clinical use, the power consumption of the isolated section needs to be minimal.
As small dimensions reduce the capacitance of the isolated section to the environment [3],[4], another important goal was to minimize as far as possible the dimensions of the isolated section.
II. SYSTEM SPECIFICATIONS
The system described in this paper was developed for the recording of 64 ECG channels with a high resolution. The demands in terms of noise level and dynamic range specifications were higher than for typical ECG recording systems: the resolution of the system had to be sufficiently high to allow measurements of small amplitude signals, e.g. late potentials or body surface His-Purkinje signals . The measured ECG signals were to be presented as Body Surface Map recordings (potential distributions over the body) ,,, which necessitated easy interfacing to the personal computer used to compute the maps. The bandwidth required was 100 Hz per channel and in this bandwidth the transmission and receiver circuits were not to add significant noise to the 0.4 æVrms equivalent input noise generated by the preamplifiers (approx. 2 æVpk-pk). A dynamic range of 75 dB was specified to allow for input signals up to 12 mVpk-pk. In view of the requirements in terms of dimensions and power consumption, a transmission circuit was needed that could be implemented with a minimal number of components and with a minimal power consumption. Both analog and digital transmission formats were considered. With the components available today, an analog transmission format, i.e. pulse position modulation, would have offered the lowest possible power consumption since a suitable VCO (Voltage Controlled Oscillator) with a power consumption of approx. 10 mW is readily available. The digital equivalent - the A/D (analog to digital) converter - currently available has a power consumption which is at least ten times as high. However, a digital transmission format offers a better signal to noise ratio, a better channel separation, a higher IMRR [4],[5], and easier interfacing to a computer. In addition, A/D converters with a lower power consumption may become available in the years ahead, which will allow easy upgrading of a digital system. Therefore, it was decided to utilize a digital transmission format with 14 bit accuracy. The usual set-up in interfaces to digital systems is to employ a "handshake" procedure: data is transmitted to the computer, whereas control and timing information is transmitted to the isolated section. In our application, this would call for the use of at least two fibers as well as optical transmitters and receivers in both the isolated section and the signal processing section. It is not attractive to use an optical receiver in the isolated section because of its relatively high power consumption (> 100 mW) caused by the large gain-bandwidth product required. To allow for the application of a single optical fiber, we devised a transmission format suitable for a data link with transmission in one direction over a single transmission path. Finally, the receiver had to be suitable to interface to a commercially available DMA (Direct Memory Access) board for use in a standard personal computer (IBM compatible).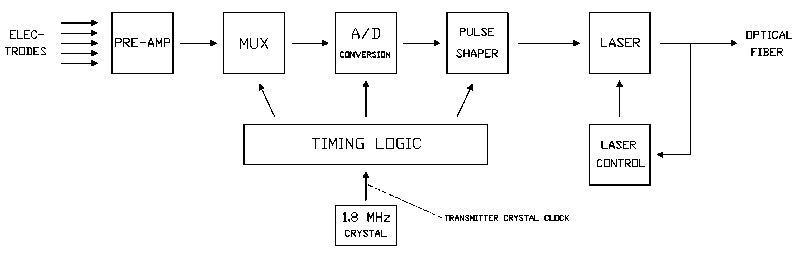
Fig. 1 : Block diagram of the transmitter.
III. THE TRANSMITTER
A block diagram and simplified schematic of the transmitter are shown in Figs. 1 and 2. Amplification of the bioelectric signals is performed by a low-power 64 channel preamplifier described in a previous paper (gain 54 dB). The preamplifier outputs (bandwidth 100 Hz) are time-multiplexed to form a single transmission channel. A sample rate of 1 kHz per channel (oversampling) was used to relax the specifications for the anti-aliasing low-pass filtering.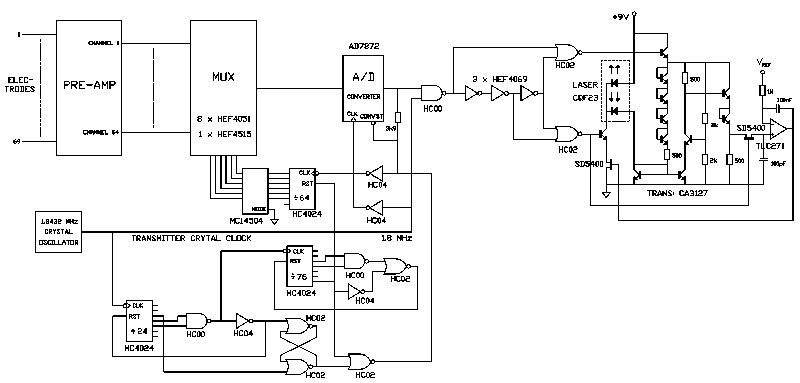
Fig. 2 : Simplified schematic diagram of the transmitter
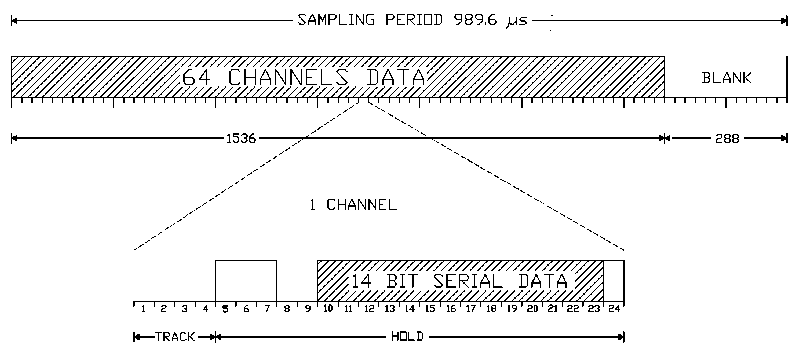
The serial output of the A/D converter (Analog Devices AD7872) is fed to the control circuit of a solid state laser diode (Philips CQF23). The fast response of the laser allowed for the transmission of very short infra-red light pulses of 20 ns. One short pulse is transmitted for each high-level period, resulting in a duty-cycle of 1.4%. A feedback control circuit ensures a constant optical output of the laser during the pulse . Commercially available high speed LED's may form an alternative for laser diodes. However, because of their inefficient coupling to the fiber ,, only a few tens of æW optical power coupled into the fiber can be achieved at drive currents compara- ble to the currents needed to drive the laser diode. With the laser diode, nearly all of the optical output (2 mW at 50 mA drive current) is coupled into the fiber, which simplifies the design of the receiver and makes the system much more tolerant to poor fiber connections and dirty fiber terminations. A minimal power consumption is achieved by using high speed CMOS (HC/HCT series) for all logic functions and by designing the circuit so as to minimize the switching-frequencies of the logic circuits involved (the power consumption of CMOS logic is proportional to the switching frequency). Interference between the digital and analog sections of the circuit are minimized by using separate power supplies for both sections and by a shield between the circuits. The shields in the cabinet as well as the cable shields (all 68 input cables are shielded) are driven with a low impedance version of the common mode signal (guarding) in order to keep the common mode input impedance as high as possible [3]. The exterior of the cabinet is made of plastic to preclude galvanic contact between the patient and the driven shield. In addition, the current output of the shield driver is limited to 10 æA to prevent excessive current flow through the patient in case of a defective cable isolation.

IV. THE RECEIVER
A block diagram and simplified schematic diagram of the receiver are shown in Figs. 4 and 5. The incoming light pulses are converted to a TTL compatible signal by a fast p-i-n photodiode (Philips BPF40), an integrated transimpedance amplifier (NE5212) and a fast comparator (NE529).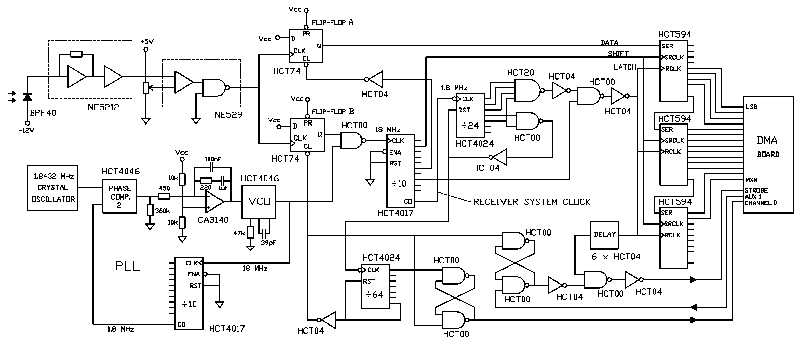
The tracking of the receiver with the transmitter is based on the frequency equality of the clock circuits in the transmitter and the receiver. For the system described, two independent clock circuits were required which had to track better than one tenth of a clock cycle over one sampling interval of 1536 clock cycles (frequency tolerance: +/- 70 ppm). This condition is met by two identical 1.8432 MHz crystal oscillator circuits provided both circuits are within the temperature range of 0 to +50 degrees Centigrade.
A PLL (Phase Locked Loop) is used to multiply the frequency of the crystal oscillator in the receiver by a factor of ten (a factor of less than eight would introduce too much jitter whereas the PLL does not function properly above 20 MHz). The resultant 18 MHz signal is used to synchronize the circuits in the transmitter and receiver.
The first incoming pulse after a blank triggers flip-flop B (see Figs. 4 and 5) and this event defines the beginning of a sampling interval in the receiver. As soon as the output of flip-flop B becomes high, the 18 MHz output signal of the PLL is passed to a divide-by-ten circuit. The first rising edge of the 18 MHz signal that is passed to the divide-by-ten circuit determines the phase of the 1.8 MHz output signal of the divide-by-ten circuit (the "receiver system clock" in Figs. 4 and 5). This last signal remains approximately in phase with the clock in the transmitter (the "transmitter crystal clock" in Figs. 1 and 2) for the next 1536 clock cycles. After staying "high" during 1536 receiver system clock cycles, flip-flop B is reset and the receiver logic, except for the free-running 1.8 MHz crystal clock and coupled PLL, waits for the first pulse of the next sampling interval.
During the first clock cycles of a sampling interval, the receiver system clock is never more than one tenth of a clock cycle out of phase with the transmitter crystal clock. The phase difference between the transmitter crystal clock and the receiver system clock after say 1500 clock cycles (the last few channels of the sampling interval), may increase to a maximum of two tenths of a clock cycle due to a possible small difference in frequency between the crystals in the transmitter and the receiver. After the system is switched on, the receiver takes the first incoming pulse - which at that moment can be any arbitrary pulse in the sampling interval - as the first pulse of a sampling interval. This first incoming pulse triggers flip-flop B which is reset 1536 clock cycles later. Because a full sampling interval lasts 1824 cycles, each next sample, a pulse at least 288 cycles (the length of the blank) earlier in the sampling interval will be perceived as the first pulse. After at the most 6 samples (6 x 288 > 1536) flip-flop B is reset during the blank at the end of a sampling interval. From then on flip-flop B is triggered by the first incoming pulse after each blank and the transmitter and receiver are synchronized.
The incoming pulses are lengthened by flip-flop A and transferred to a shift register. The parallel data in the shift register is read by a commercially available DMA board (MetraByte PDMA-32) and stored into the memory of a personal computer (IBM compatible).
V. RESULTS
The key specifications of the complete system are listed in Table 1. The total power consumption of the isolated section of the system is 210 mW. The A/D converter dissipates approx. 75 % of the total battery power. For comparison, the specifications of a typical commercially available 6-channel ECG recording system are given in the second column of Table 1. The preamplifiers and 90% of the digital circuitry in the isolated section are constructed using the SMD (Surface Mount Devices) production technique. All parts used are commercially available. The most expensive components in the system are the semiconductor laser ($ 100), the optical fiber ($ 100) and the A/D converter ($ 70). Photographs of the isolated section, the receiver and a 64 radiotransparent electrode set , are shown in Figs. 6a and 6b.The system is being used on a daily basis to evaluate the clinical significance of Body Surface Map measurements. Since the isolated section operates 10 hours on a set of batteries (three 9 V rechargeable batteries, size PP3, capacity 110 mAh) and since the batteries can be changed in less than 30 seconds, the battery power supply of the isolated section presented no difficulties in day to day clinical use. Usually optical fibers with a length of 5 - 10 m are used, but fiber lengths up to 100 m will not deteriorate the recordings (50/125 æm graded index silica fibers were used). The susceptibility to interference has proved to be much lower than with a previous Body Surface Map recording system [3],,, of which the isolation capacitance was much higher. An example of one of the recordings is shown in Fig. 7.
Table I: System specifications | |
Equivalent input noise voltage (æVrms, 0.1-100 Hz) | 0.4 |
Equivalent input noise current (pArms, 0.1-100 Hz) | 5 |
Bandwidth per channel (+0/-3 dB) (Hz) | 0.16-100 |
Differential AC input range (mVpk-pk) | 12 |
Dynamic range (dB) | 72 |
Sample rate (Hz per channel) | 1010.5 |
Common mode rejection ratio (dB at 50 Hz) | 119 |
Isolation mode rejection ratio2 (dB at 50 Hz) | > 166 |
Leakage current3 (æArms), typical | < 2 |
Maximum | 6 |
Isolation capacitance5 (pF), typical | < 30 |
Maximum | 90 |
Isolated section, power consumption (mW) | 210 |
Size (mm) | 52 x 101 x 269 |
Weight including batteries (g) | 1215 |
Receiver, power consumption (mW) | 580 |
Size (mm) | 42 x 103 x 175 |
Weight (g) | 410 |
VI. CONCLUSION
A 64 channel measurement system is presented which offers a virtually ideal patient isolation during multichannel bioelectric measurements. For the larger part, this was due to the use of an optical fiber as the only link across the isolation barrier and to a minimal size of the isolated section.The combination of a low-noise pre-amplifier, the extensive application of guarding techniques, the use of a 14-bit digital transmission format and an optimal isolation provided ECG recordings with a very low level of noise and interference: in practice, the noise produced by the electrodes and various bioelectric signals not related to the heart appeared to be the limiting factor. The consistent low-power design of the isolated section made a power supply with small sized batteries a practical possibility. Only when A/D converters with a lower power consumption become available, will a further reduction of the power consumption of the isolated section be possible.
Hiç yorum yok:
Yorum Gönder